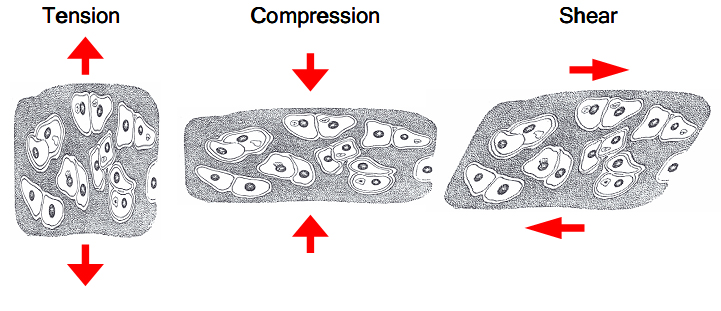
Understanding the interplay between physics and biophysics offers fascinating insights into how fundamental physical principles shape the complexities of biological systems. From the laws of thermodynamics guiding cellular energy production to quantum mechanics optimizing photosynthesis, this chapter explores the cross-disciplinary nexus that illuminates life at a molecular and systemic level. Through these explorations, we see how the same physics concepts that govern inanimate matter also provide a framework for understanding the dynamic processes within living organisms.
This series delves into specific examples where physics principles reveal the mechanisms behind biological phenomena. It discusses how the laws of thermodynamics explain energy transformations in cells, how molecular motors convert chemical energy into mechanical work, and how quantum mechanics enhances our understanding of photosynthesis. Additionally, statistical mechanics is applied to population genetics, showing how physicists’ tools can predict genetic evolution. By bridging the gap between physics and biology, we’ll uncover how these interactions advance both fields and open new pathways for scientific discovery.
Role of Physics in Biology
Physics plays a pivotal role in deepening our understanding of biological processes, offering profound insights into the intricacies and mechanisms that sustain life. One foundational concept is how physical laws like thermodynamics control how cells generate energy.
Thermodynamics, particularly the laws governing energy conservation and transformation, is crucial for explaining cellular metabolism. Cells rely on the conversion of chemical energy from nutrients into usable forms. This process, known as cellular respiration, can be broken down into stages where energy is produced, utilized, and conserved. The first law of thermodynamics, which states that energy cannot be created or destroyed but only transformed, underpins this entire metabolic pathway. For instance, during glycolysis and the citric acid cycle, glucose is oxidized, gradually releasing energy stored in its chemical bonds. This energy is then captured in molecules like ATP, which cells use to power various functions. The second law of thermodynamics, focusing on entropy, explains why some of the energy is always lost as heat, making complete efficiency unattainable but driving systems towards equilibrium.
Diving deeper into the cell’s machinery, we encounter molecular motors—tiny protein structures that convert chemical energy into mechanical work. These include kinesin and dynein, which move along microtubules, and myosin, which travels along actin filaments. Their function is essential for cellular locomotion, intracellular transport, and muscle contraction. Take myosin, for example; it uses ATP hydrolysis to generate force and move along actin filaments, facilitating muscle contractions. Each step involves a conformational change in the myosin molecule, a perfect illustration of how mechanical principles can explain biological actions at the microscopic level. Understanding these mechanics allows scientists to decipher how cells organize their components and respond to external stimuli.
Another fascinating intersection between physics and biology is seen in photosynthesis, the process by which plants convert light energy into chemical energy. Quantum mechanics offers a compelling explanation for the remarkable efficiency of this process. Photosynthetic organisms have evolved to utilize quantum coherence, enabling them to maintain multiple potential pathways for energy transfer simultaneously. This phenomenon ensures that energy is transferred to reaction centers with minimal loss, optimizing the conversion process despite fluctuating light conditions. The idea that quantum principles, usually associated with subatomic particles, apply to a macroscopic biological system underscores the sophistication of natural processes and challenges researchers to rethink traditional boundaries between disciplines.
In population genetics, statistical mechanics provides powerful tools for modeling the dynamics of gene frequencies within populations. Just like how statistical mechanics deals with large numbers of particles and their interactions to predict macroscopic properties in physics, it helps geneticists understand how evolutionary forces such as mutation, selection, genetic drift, and migration affect population structure over time. By applying principles like probability distributions and mean field approximations, scientists can predict the behavior of alleles in a given environment. This approach has been instrumental in studying phenomena like the spread of advantageous traits or the impact of genetic bottlenecks, providing a quantitative framework for evolutionary biology.