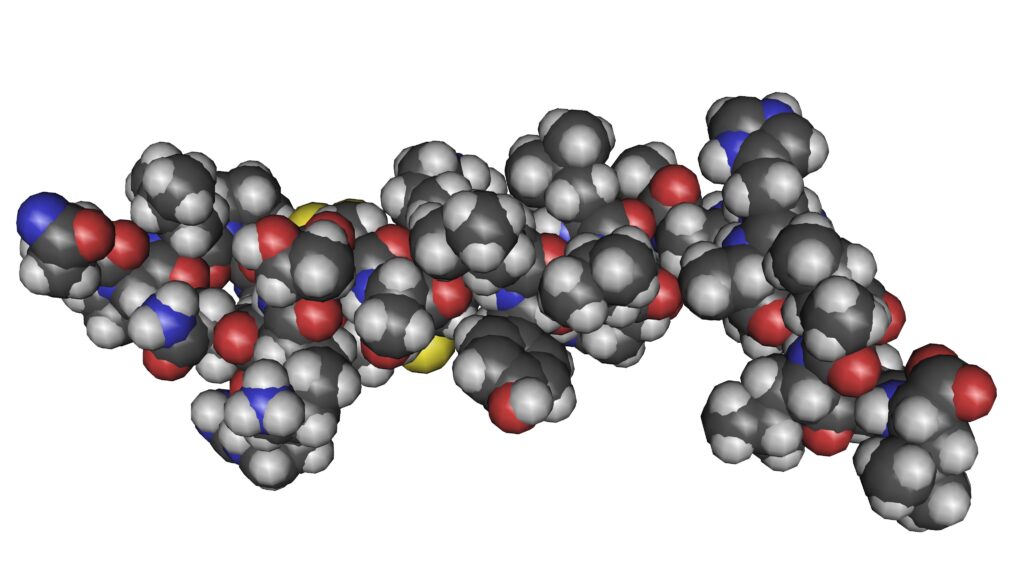
In the realm of biophysics, understanding the physical properties of biomolecules is crucial to grasp how biological systems function at a molecular level. This subpoint will delve into several key concepts that illustrate this interplay, beginning with protein folding driven by hydrophobic interactions.
Proteins are essential molecules within living organisms, performing a wide range of functions from catalyzing metabolic reactions to providing structural support. The process of protein folding, where a polypeptide chain folds into its functional three-dimensional structure, is predominantly influenced by hydrophobic interactions. Hydrophobic amino acids, which repel water molecules, tend to cluster together in the interior of the protein, away from the aqueous environment. This drive to minimize exposure to water helps stabilize the folded structure. Misfolding of proteins can lead to diseases such as Alzheimer’s and Parkinson’s, highlighting the significance of correct protein folding mechanisms.
Next, we examine the helical structure of DNA, a molecule that holds the genetic blueprint for life. The iconic double helix configuration of DNA is largely maintained by hydrogen bonds between complementary base pairs—adenine with thymine, and guanine with cytosine. These hydrogen bonds form the rungs of the helical ladder, while the sugar-phosphate backbone constitutes the sides. Despite being relatively weak on their own, the collective strength of multiple hydrogen bonds provides stability to the DNA molecule, ensuring accurate replication and transcription processes that are vital for cellular function and inheritance.
Another fundamental structure in biology is the lipid bilayer, which forms the basis of cell membranes. Lipid molecules have a hydrophilic (water-attracting) head and hydrophobic (water-repelling) tail. When in an aqueous environment, these molecules arrange themselves into a bilayer with the hydrophobic tails facing inward, shielded from water, and the hydrophilic heads facing outward, interacting with the aqueous surroundings. This arrangement creates a semi-permeable membrane that controls the movement of substances in and out of the cell, maintaining homeostasis. The fluidity of the lipid bilayer is also crucial for the proper functioning of membrane proteins, which facilitate communication and transport across the cell membrane.
Furthermore, RNA molecules, akin to DNA, play critical roles in protein synthesis and gene regulation. The secondary structure of RNA is stabilized by thermodynamic factors. For instance, RNA molecules can fold into various structures like hairpins and loops through intramolecular base pairing. The thermodynamic stability of these structures depends on the sequence of bases and environmental conditions such as temperature and ionic concentration. Stable RNA structures are necessary for their diverse functions, including carrying genetic information from DNA to ribosomes for protein synthesis, regulating gene expression, and catalyzing biochemical reactions as ribozymes.
Understanding these physical properties provides a rich insight into the complex mechanisms governing biological molecules. The principles of physics offer valuable tools to predict and manipulate these properties, further advancing our comprehension of life’s molecular underpinnings.
To continue exploring protein folding, it’s fascinating to consider how experimental techniques like X-ray crystallography and nuclear magnetic resonance (NMR) spectroscopy have allowed scientists to unravel the intricate details of protein structure. These methods reveal the atomic-level arrangement within proteins, shedding light on how specific amino acid sequences determine the overall shape and stability of the molecule. Furthermore, computational models and simulations, grounded in physical laws, enable researchers to predict protein folding pathways and the effects of mutations on protein stability and function.
Transitioning back to the helical structure of DNA, the role of hydrogen bonding cannot be overstated. It not only ensures the fidelity of genetic information during replication but also plays a pivotal role in the regulation of gene expression. For example, the formation of hydrogen bonds can influence the binding affinity of transcription factors and other regulatory proteins, thereby controlling the accessibility of certain genes for transcription. Additionally, the flexibility of the DNA helix allows it to bend and supercoil, facilitating the packaging of long DNA strands into compact chromatin structures within the nucleus of eukaryotic cells.
As for lipid bilayers, their dynamic nature is essential for various cellular processes. One prominent example is endocytosis, where the cell membrane engulfs external materials to bring them into the cell. The fluidity of the lipid bilayer permits the membrane to deform and form vesicles, encapsulating the material to be transported. Similarly, in exocytosis, vesicles containing cellular products fuse with the plasma membrane to release their contents outside the cell. These processes are integral to nutrient uptake, signal transduction, and waste removal, highlighting the critical role of the lipid bilayer’s physical properties in maintaining cell functionality.
Additionally, the study of RNA secondary structures has expanded our understanding of non-coding RNAs, which do not encode proteins but perform regulatory functions. MicroRNAs (miRNAs) and small interfering RNAs (siRNAs), for example, regulate gene expression by binding to target messenger RNAs (mRNAs) and promoting their degradation or inhibiting their translation. The ability of these RNA molecules to form stable secondary structures is essential for their interaction with other biomolecules and their effectiveness in gene regulation. Understanding the thermodynamic principles that govern these interactions provides a foundation for developing RNA-based therapeutics, such as small interfering RNA (siRNA) treatments for silencing disease-causing genes.